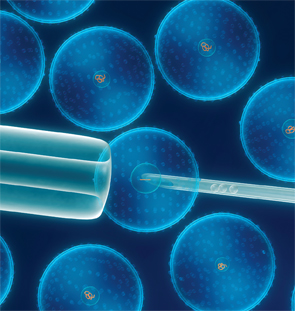
The National Academy of Sciences has convened a panel of experts to assess current regulations and recommend what additional oversight will be needed with regard to gene manipulation.
Image Credit: Sebastian Kaulitzki/shutterstock.com
Every so often, a major scientific breakthrough profoundly alters the trajectory of scientific research. In the 1960s, microbiologists sparked the recombinant-DNA revolution with the discovery that bacteria have innate immune systems based on restriction enzymes. These enzymes bind and cut invading viral genomes at specific short sequences, and scientists rapidly repurposed them to cut and paste DNA in vitro, transforming biologic science and giving rise to the biotechnology industry.1
In the 1970s, the seminal efforts of the late Nobel laureates, César Milstein, PhD, and Georges Köhler, PhD, in the Laboratory of Molecular Biology in Cambridge, UK, demonstrated the efficacy of monoclonal antibodies as probes to investigate the pathological pathway of neurological conditions. Although limited in scope, this initial application paved the way for the adoption of monoclonal antibodies as diagnostic tools. Perhaps more importantly, other investigators recognized their potential in the therapeutic sphere. Within years of their discovery, monoclonal antibodies served as an invaluable platform in the development of biologic therapies for rheumatic diseases.2 (See Rheuminations; January 2012, “A Short History of RA Therapeutics.”)
In the 1980s, using the polymerase chain reaction (PCR), we learned how to speedily amplify specific DNA sequences, creating a way to generate large amounts of genetic material from a single cell, a previously laborious technique.
More recently, a fascinating discovery that allows for the easy manipulation of an organism’s genome has rocked the world of biology. Unlike some of the other scientific breakthroughs described earlier, this one has the potential to alter our genomes, and so the use or misuse of this technology will have profound implications for society.
CRISPR: From the Yogurt Cup to the Human Genome
More than 30 years ago, scientists observed a strange pattern in some bacterial genomes: One DNA sequence would be repeated over and over again, while unique sequences would be found in between the repeats. This odd configuration, coined clustered regularly interspaced short palindromic repeats (CRISPR), was subsequently found to serve as a naturally occurring, ancient defense mechanism in a wide range of bacteria. How does it work?3
CRISPR, in combination with a set of enzymes called Cas9 (CRISPR-associated proteins), uses a guide RNA molecule to home in on its target DNA, then edits it by inserting desired sequences or disrupts it altogether. To use an analogy, these lab-made CRISPR systems are the cellular version of a word processor’s find-and-replace function.
About 15 years ago, a couple of food microbiologists observed that following infection the bacterial genome of certain streptococcal species integrated a sequence of the viral genome, called a spacer. This sequence served as a guide for destroying any matching DNA so that subsequent viral infections were fended off. Bacteria use this system naturally, and dairy food scientists wanted to harness it to immunize bacterial starter cultures against phage infection, a huge source of product contamination, affecting 2% of cheese production worldwide.4 In 2012, the DuPont Chemical company of Wilmington, Del., the patent holder of CRISPR-enhanced starter cultures, announced its first commercial application that could make pizza cheese consistently taste the same. It dubbed the blend of S. thermophilus strains that were used, CHOOZIT SWIFT. Given DuPont’s share of the dairy culture market, estimated to be about 50%, the chances are quite good that you have already eaten CRISPR-modified food.4 Buon appetito!
CRISPR/Cas9 is considered to be among the simplest genome-editing tools to work with because it relies on RNA–DNA base pairing, rather than the engineering of proteins that bind particular DNA sequences. This has transformed the field overnight. It used to take at least three generations of progeny to create a knockout mouse carrying the altered genetic material; with CRISPR, both gene copies are edited simultaneously, so a knockout rodent can be created in a single generation. The process has become so straightforward that an edited gene can be produced in a matter of days.
“In the past, it was a student’s entire PhD thesis to change one gene,” remarks Bruce Conklin, MD, professor of medicine at the University of California in San Francisco. “CRISPR just knocked that out of the park.”5
Talk about speed: Cas9 must identify a short three-base-pair DNA sequence immediately following the primer sequence, dubbed PAM, which occurs about 300 million times within the human genome. For it to be effective though, it must work at lightning speed, because recent studies have indicated that as many as 300,000 bindings may occur before the correct sequence of DNA is cut.6
Researchers have traditionally relied heavily on model organisms, such as mice and fruit flies, partly because they were the only species that came with a good toolkit for genetic manipulation. Now CRISPR is making it possible to edit genes in many more organisms. Researchers have begun using CRISPR to develop better biofuels and to create new enzymes for industrial markets, where they are used in laundry detergents, water treatment and paper milling. In agriculture, companies are using the system to make crops more resistant to pests and drought, without using genes spliced in from other species, such as a flounder gene inside a tomato. Livestock breeders can harness it to produce animals with more muscle mass and leaner meat, faster and more predictably than with ordinary crossbreeding.5
Yet these are all relatively straightforward adaptations of this technology. What happens when we start using CRISPR as a tool for human genome editing?1 For example, to treat human immunodeficiency virus (HIV) infection, physicians might edit a patient’s immune cells to delete the CCR5 gene, conferring the resistance to HIV carried by the 1% of the U.S. population lacking functional copies of this gene. For patients becoming progressively blind due to the genetically dominant forms of retinitis pigmentosa, the mutant allele in retinal cells might be inactivated. Patients at high risk for cardiovascular events because they are homozygous for familial hypercholesterolemia may one day undergo liver cell editing that could restore a functional copy of the gene encoding low-density lipoprotein receptors. Similarly, editing of blood stem cells might one day cure sickle cell anemia and hemophilia. Could harmful genes, such as BRCA, that confer a heightened risk for cancer be deleted from a child carrying this repertoire? Aldous Huxley must be rolling over in his grave.
Driving Genes
For all the benefits that CRISPR promises, there are some significant consequences to the use of this technology that society must consider carefully. Usually, a genetic change in one organism takes a long time to spread through a population. In 2003, an evolutionary biologist, Austin Burt, PhD, professor of evolutionary genetics at the Imperial College in London, England, outlined an ingenious way to flout the laws of both inheritance and evolution.7 For more than a century, geneticists have known that in organisms that pair up to reproduce, most genes have a 50–50 chance of being inherited, as Moravian scientist and Augustinian friar Gregor Mendel famously demonstrated using pea plants. Similarly, evolutionary biologists have known since Charles Darwin that natural selection eliminates inherited traits that reduce an organism’s fitness, such as a mosquito’s susceptibility to insecticide.
Dr. Burt described how gene drive can outsmart both Mendel and Darwin. In nature, he noted, some genes copy themselves into multiple places in a genome. Like a dishonest candidate stuffing the ballot box, these extra copies increase a gene’s odds of “winning,” that is, being inherited. In addition, other genes can destroy related ones, also ensuring their transmission into the next generation.
Although this natural gene drive is unpredictable and scattershot, Dr. Burt showed that certain tricks of molecular biology could achieve the same results—causing a gene to be inherited by many more organisms through many more generations than standard genetics and natural selection allow. His theory, published in 2003, lay dormant because there was no tool available that could put this theory to practice. But now, instead of genetically engineering single organisms, gene drive can sweep an edited gene exponentially faster through a population by allowing a mutation made by CRISPR on one chromosome to copy itself to its partner in every generation, so that nearly all offspring will inherit the change. The genetics of vast populations are immediately altered. For example, a mutation engineered into a mosquito strain that carries the malaria parasite could spread through a large population within a season. If that mutation reduced the number of offspring a mosquito produced, then the population could be wiped out, along with any malaria parasites being carried. In fact, a recent report described a working prototype for this experiment.8
As great as this scenario may sound, once nature is tampered with, there may be no turning back should things go awry. Altering an entire population of mosquitos, or eliminating them altogether, could have drastic and unknown consequences for an ecosystem: It might mean that other pests emerge, for example, or it could affect predators higher up the food chain. Hypothetically, a guide RNA could mutate over time such that it targets a different part of the genome. This mutation could then race through the population, with unpredictable effects.9,10 Not only could gene drives doom species to extinction or change the course of evolution, bioterrorists could alter the genomes of disease-causing organisms to make them more lethal or more infectious and use gene drives to spread that trait throughout a population. Someone with nefarious intentions and $10,000—the estimated cost of materials to construct a gene drive—can wreak considerable havoc. This has caught the attention of the United Nations office that oversees the biological weapons treaty, as well as that of the FBI’s Weapons of Mass Destruction directorate.10
In an effort to identify possible ramifications of gene drives, the National Academy of Sciences has convened a panel of experts to assess current regulations and recommend what additional oversight will be needed.
Designer Humans
Can CRISPR excise deleterious genes from our genome? It is one thing for scientists to genetically modify bacterial cultures so that our yogurt tastes fresher or our cheese pizza is tastier, but what about modifying genes that control risks for certain cancers, infections or even autoimmune diseases? What about editing embryos?
This latter possibility ratchets things up a few notches, because germ line, unlike somatic cell modifications, are heritable, and they could have an unpredictable effect on future generations. In a world first, Chinese scientists have recently reported editing the genomes of human embryos.11 The researchers tried to head off ethical concerns by using “non-viable” embryos obtained from local fertility clinics that were carrying an extra set of chromosomes, which prevented maturation and live birth. They studied the ability of the CRISPR/Cas9 system to edit a gene called HBB, which encodes the human β-globin protein. Mutations in the gene are responsible for β-thalassemia.
A fascinating discovery that allows for the easy manipulation of an organism’s genome has rocked the world of biology. [It] has the potential to alter our genomes, & so the use or misuse of this technology will have profound implications for society.
The team injected 86 embryos. Of the 71 embryos that survived, 54 were genetically tested. This revealed that just 28 were successfully spliced and that only a fraction of those contained the replacement genetic material. Most worrisome, they observed a surprising number of off-target mutations assumed to be introduced by the CRISPR/Cas9 complex acting on other parts of the genome. This effect is one of the main safety concerns surrounding germ line gene editing, because these unintended mutations could be harmful. The rates of such mutations were much higher than those observed in gene-editing studies of mouse embryos or human adult cells. And the investigators conceded that they likely detected just a fraction of the unintended mutations because their study looked only at a portion of the genome, known as the exome.
Brave New World indeed.
Simon M. Helfgott, MD, is associate professor of medicine in the Division of Rheumatology, Immunology and Allergy at Harvard Medical School in Boston.
References
- Lander ES. Brave new genome. N Engl J Med. 2015 Jul 2;373(1):5–8.
- Marks L. A healthcare revolution in the making: The story of César Milstein and monoclonal antibodies.
- How does CRISPR work? Stat News. 2015 Nov 6.
- Grens K. There’s CRISPR in your yogurt. The Scientist. 2015 Jan 1.
- Kahn J. The Crispr quandary. The New York Times. 2015 Nov 9.
- Knight SC, Xie L, Deng W, et al. Dynamics of CRISPR-Cas9 genome interrogation in living cells. Science. 2015 Nov 13;350(6262):823–826. doi: 10.1126/science.aac6572.
- Burt A. Site-specific selfish genes as tools for the control and genetic engineering of natural populations. Proc Biol Sci. 2003 May 7;270(1518):921–928. doi: 10.1098/rspb.2002.2319.
- Gantz VM, Jasisnskiene N, Tatrenkova O, et al. Highly efficient Cas9-mediated gene drive for population modification of the malaria vector mosquito Anopheles stephensi. PNAS. 2015 Oct;112(49):E6736–E6743.
- Ledford H. CRISPR, the disruptor. Nature. 2015 Jun 4;522(7554):20–24. doi: 10.1038/522020a.
- Begley S. Gene drive gives scientists power to hijack evolution. Stat News. 2015 Nov 17.
- Cyranoski D, Reardon S. Chinese scientists genetically modify human embryos. Nature. 2015 Apr 22.